The Science behind the Modern Solar Cell
- saratahir19
- Feb 6, 2017
- 4 min read
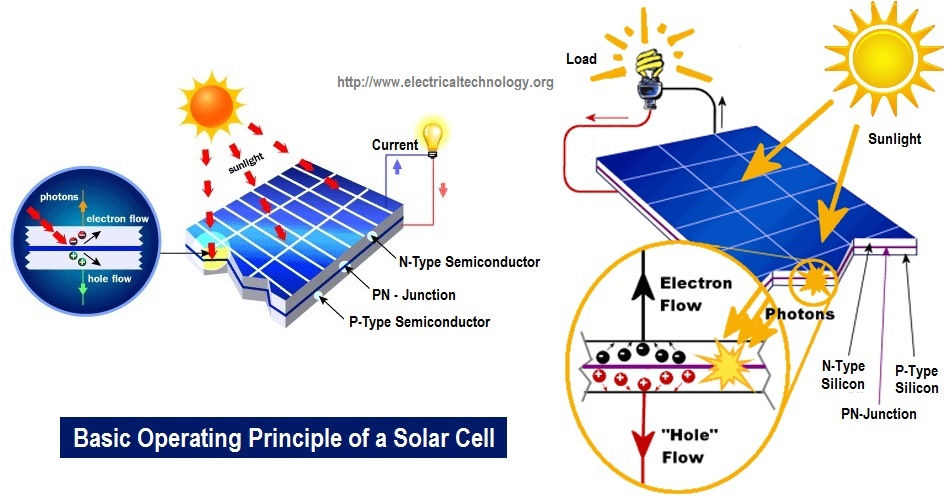
Solar energy is one of our key sources of renewable energy, but how does it work and how is it created from solar panels?
Let’s start from the basics.
Light at its fundamental level is comprised of photons. What essentially occurs in solar panels is the photons from the sun hit the silicon atoms of the solar cells, triggering an energy transfer to loose electrons that ultimately get knocked off.
Solar panels are comprised of tiny solar cells where all the action occurs. These solar cells are also called photovoltaic (PV) cells, “photo” meaning light and “voltaic” meaning electricity. When light strikes the cell, a certain portion of the light is absorbed within the semiconductor material. Basically, the energy of the absorbed light is transferred to the semiconductor, typically comprised of Silicon. This energy knocks electrons loose, allowing a free flow of electrons.
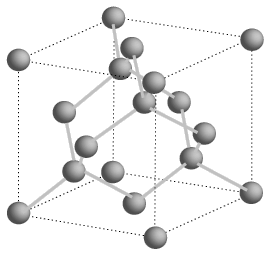
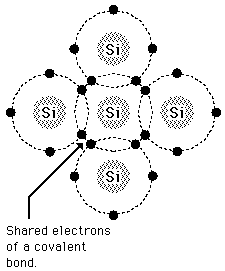
Silicon is an important component of solar cells. Why? Put frankly, Silicon is a semiconductor (it is able to conduct electricity). Unlike a typical metal, however, silicon gets better at conducting electricity as temperature increases. Silicon has some special chemical properties in its crystalline form. An atom of silicon has 14 electrons, arranged in three different shells. The first two shells hold two and eight electrons respectively and are completely full. The outer shell, however, is only half full with just four electrons. A silicon atom will always look for ways to fill up its last shell, and to do this, it will share electrons with four nearby atoms. This forms a crystalline structure that is vital to PV cells.
However, there is a problem with pure crystalline silicon: it is a poor conductor. To fix this problem, the silicon in a solar cell is usually accompanied with other atoms which changes the way they work drastically. Consider silicon with an atom of phosphorous incorporated in random locations, and maybe one for every million or two silicon atoms. Phosphorous has five electrons in its outer shell, not four. It still bonds with its silicon neighbor atoms, but in a sense, the phosphorous has one electron that doesn't have anyone to hold hands with. It doesn't form part of a bond, but there is a positive proton in the phosphorous nucleus holding it in place. This process of adding impurities on purpose is called doping. And when doped with phosphorous, the resulting silicon is called N-type ("n" for negative) because of the prevalence of free electrons. You guessed it, N-type doped silicon is a much better conductor than pure silicon. Why? Keep reading.
When energy is added to pure silicon it can cause some electrons to leave their atoms by breaking free from their bonds. A hole is left behind in each case as electrons break free. These electrons, called free carriers, wander randomly around the crystalline lattice carrying an electrical current looking for another hole to fall into. However, there are so few of them in pure silicon, that they aren't very useful.
But in impure silicon with phosphorous atoms mixed in, something different happens. It takes a lot less energy to knock loose one of our "extra" phosphorous electrons because they aren't tied up in a bond with any neighboring atoms. As a result, most of these electrons do break free, and we have a lot more free carriers than we would have in pure silicon.
There are usually two parts of a solar cell. The other part of a typical solar cell is doped with the element boron, which has only three electrons in its outer shell instead of four, to become P-type silicon. Instead of having free electrons, P-type ("p" for positive) has free openings and carries the opposite (positive) charge.
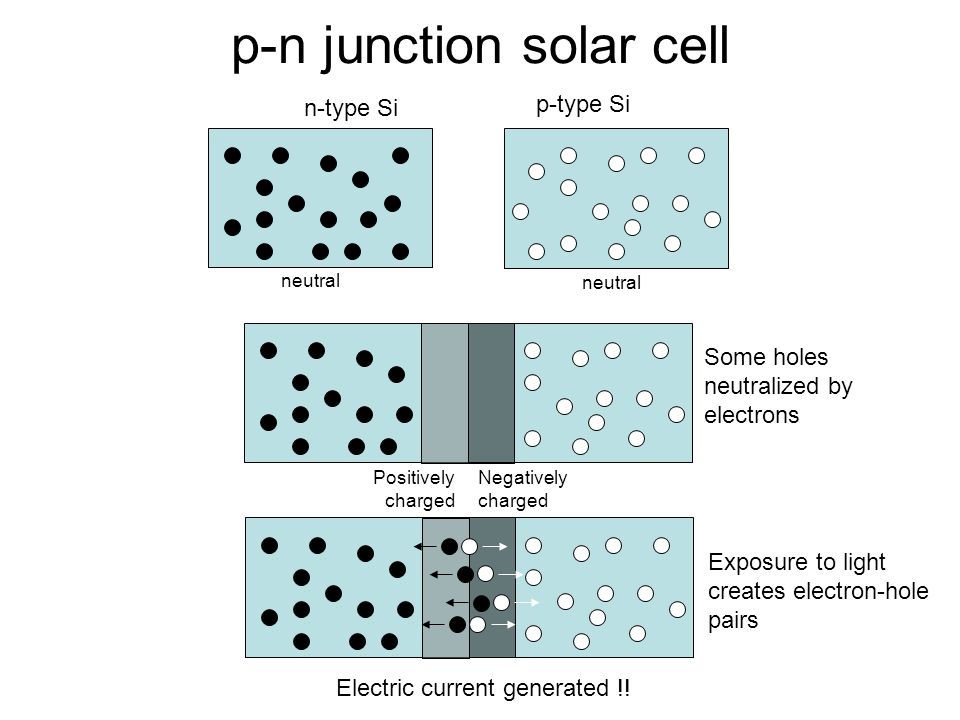
Before putting both parts of the solar cell together (the negative and positive parts), the separate parts were electrically neutral. When you put the two parts together, an electric field is formed. Suddenly, the free electrons on the N side see all the openings on the P side, and there's a mad rush to fill them. Do all the free electrons fill all the free holes? No. If they did, then the whole arrangement wouldn't be very useful. However, right at the junction, they do mix and form something of a barrier, making it harder and harder for electrons on the N side to cross over to the P side. Eventually, equilibrium is reached, and we have an electric field separating the two sides.
This electric field acts as a diode, allowing (and even pushing) electrons to flow from the P side to the N side, but not the other way around. It's like a hill -- electrons can easily go down the hill (to the N side), but can't climb it (to the P side).
When light, in the form of photons, hits our solar cell, its energy breaks apart electron-hole pairs. Each photon with enough energy will normally free exactly one electron, resulting in a free hole as well. If this happens close enough to the electric field, or if free electron and free hole happen to wander into its range of influence, the field will send the electron to the N side and the hole to the P side. This causes further disruption of electrical neutrality, and if we provide an external current path, electrons will flow through the path to the P side to unite with holes that the electric field sent there, doing work for us along the way. The electron flow provides the current, and the cell's electric field causes a voltage. With both current and voltage, we have power, which is the product of the two.
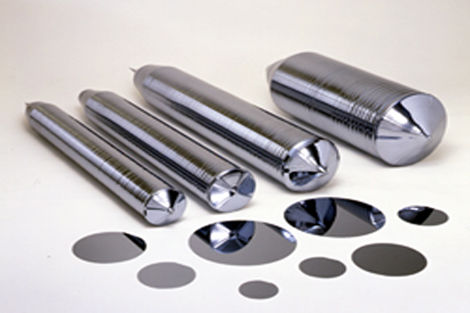
There are a few more components left before we can really use our cell. Silicon
happens to be a very shiny material, which can send photons bouncing away before they've done their job, so an antireflective coating is applied to reduce those losses. The final step is to install something that will protect the cell from the elements -- often a glass cover plate.
How much sunlight energy does our PV cell absorb? Unfortunately, probably not a lot. Click HERE to read more about the efficiency of solar cells.
Comments